Few biomedical breakthroughs have produced such a rapid and significant impact as the emergence of CRISPR-based gene editing. It was only eleven years ago when Doudna, Charpentier, and colleagues published the first demonstration that CRISPR/Cas—an adaptive immune system in bacteria—could be repurposed for programmable gene editing in other biological systems.
Since then, CRISPR has become a foundational technology, serving as the basis for a wide range of cell-based therapies targeting cancer, hematologic disorders, vision loss, musculoskeletal disorders, and many other diseases.
![Cenk Sumen, PhD [MaxCyte]](https://www.genengnews.com/wp-content/uploads/2024/01/Cenk-Sumen-Maxcyte-458x476-circle-300x300.jpg)
CSO, MaxCyte
But, while CRISPR/Cas has brought about a boon in cell therapy research, there remains a significant bottleneck in the development and manufacturing of these modalities. Critical to CRISPR-based gene editing is the need to effectively deliver the technology’s various editing components to target cells. Doing so has become routine in academic settings.
However, scaling the process to meet the demands of industrial therapeutic manufacturing is a substantial logistical challenge for which there have been few good solutions. This is particularly true for therapeutics that require the ex vivo editing of patient cells, such as CAR T-cell therapies.
With millions of patients waiting to benefit from cell therapy advances, there is an urgent need for tools that alleviate logistical bottlenecks and clear the path for large-scale, high-throughput CRISPR engineering. For this, many are turning to electroporation technology.
Electroporation and CRISPR/Cas delivery
Electroporation temporarily relaxes a cell’s plasma membrane that occurs when it’s exposed to short electrical pulses. This transiently permeabilizes the membrane to transfect molecular cargo (proteins, DNA, or RNA) into the cells. In four decades, the technology has greatly advanced enabling efficient transfer of nearly any molecular cargo into the cytoplasm while maintaining cell viability and function.

As such, alternate terms have evolved to more accurately describe the utility of highly optimized short pulses of electrical energy to allow reversable alteration of the pulsed cell membrane, such as electropulsation, electropermeabilization, and electrotransformation.
Developing a safe and effective cell therapy is far from trivial, particularly when gene editing is involved. CAR T-cell therapy, for example, requires extensive preclinical screening to optimize the chimeric antigen receptor (CAR)—a process that may require the testing of many receptor variants. Then, billions of primary patient cells must be processed to finally express the optimized CAR in the right cell type while retaining both viability and safety.
Throughout all of this, researchers will need a reliable way to deliver molecular cargo to billions of precious—and sensitive—cells and to do so without unexpected side effects.
Viral vectors have long been used to deliver genes of interest to target cells for both ex vivo and in vivo cell and gene therapies. However, these methods have substantial limitations. CRISPR-based engineering relies on the delivery of both a CRISPR-associated (Cas) enzyme and an optimized guide RNA (gRNA).
Although viral capsids can be used to deliver the genetic cargo for specific applications such as knock-in mediated by homology directed repair, these approaches lack the scalability and consistency required for clinical development. Additionally, viruses come with the added risk of genotoxicity and the potential that viral components will prompt an immune response once in the host.
MaxCyte’s non-viral technology offers efficient scaling, greater process consistency, the absence of packaging constraints, and a much reduced risk of immunogenicity. Adapting this technology from its origins in the academic lab to the rigors of highly regulated clinical manufacturing cleanrooms has enabled a commercial-ready platform with consumables, reagents, software, and supply chain that are compliant with good manufacturing practices (GMP). This has been made possible with many technological innovations, including the advent of flow electroporation technology.
Flow electroporation and cell therapies
Traditional electroporation is referred to as static electroporation because most such electroporation instruments do not allow for the movement of cells to and from the electroporation chamber during the transfection process, significantly limiting the number of cells that can be processed at one time.
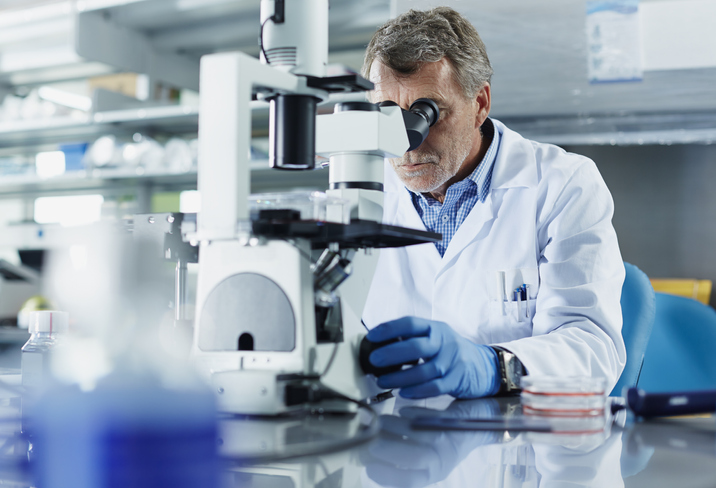
In contrast, flow electroporation technology can transfect billions of cells during a single run thanks to a process that employs a stepwise and gentle pull of cell fractions into a specially designed chamber. All cell fractions from a population are processed identically and are then pooled into one consistent batch at the end of the process.
Notably, the movement of cells through the instrument is fully automated, providing much greater scalability, process standardization, and consistency relative to static research-grade electroporation.
The benefits of scalable automation of electroporation are manifold. Because electroporation introduces molecules into the cell via temporary disruptions to the cell membrane, multiple loading agents and combinations of loading agents (e.g., mRNA plus guide RNA or RNP plus DNA) can be transfected simultaneously without adjusting the electroporation parameters. These parameters can then be universally applied regardless of the cargo.
Unlike reagent-based transfection methods, loading efficiencies with flow electroporation can be optimized simply by varying the loading agent concentrations in the electroporation reaction. This means that, with the technology’s software and interchangeable consumables, GMP-compliant protocols can be easily scaled from hundreds to billions of cells as needed. And, with automation, protocols remain robust across laboratories and users.
Flow electroporation is already enabling CRISPR-based therapies. For example, it has played a critical role in enabling Vertex to manufacture CASGEVY™ (exa-cel), the first CRISPR-based cell therapy to receive commercial approval by the MHRA in the U.K. for the potential treatment of sickle cell disease and beta thalassemia—a historic milestone in commercial CRISPR applications.
Multiple other therapies rely on CRISPR editing to improve the potency of engineered T cells by knocking out expression of endogenous T cell receptors and checkpoint inhibitors. Two years ago, a group from the University of Pennsylvania described a first-in-human, Phase I clinical trial that demonstrated the feasibility of multiplex CRISPR editing, enabled by flow electroporation technology, to improve the therapeutic efficacy of T cells that were also engineered to express a tumor-targeting T cell receptor. Similar applications are being explored in natural killer (NK) cells, macrophages, and B cells.
Flow electroporation has also been used for induced pluripotent stem cell (iPSC) engineering. As an industry use example, MaxCyte has collaborated with researchers from the Center for iPSC Research and Application (CiRA) at the University of Kyoto to generate proof-of-concept data for CRISPR-based therapies to treat musculoskeletal diseases and cancer.
As companies and scientists are pushed to develop increasingly sophisticated cellular therapies, flow electroporation technology will continue to expand access to non-viral approaches, and therapies that rely on genome editing are poised to be at the forefront of the therapeutic landscape.
While questions remain about reimbursement, durability, preconditioning regimens, off-target effects, and the long-term safety of these genome editing therapies, it seems clear that CRISPR-related technologies will continue to open new avenues for treating previously intractable diseases.
Cenk Sumen, PhD, is chief science officer at MaxCyte.